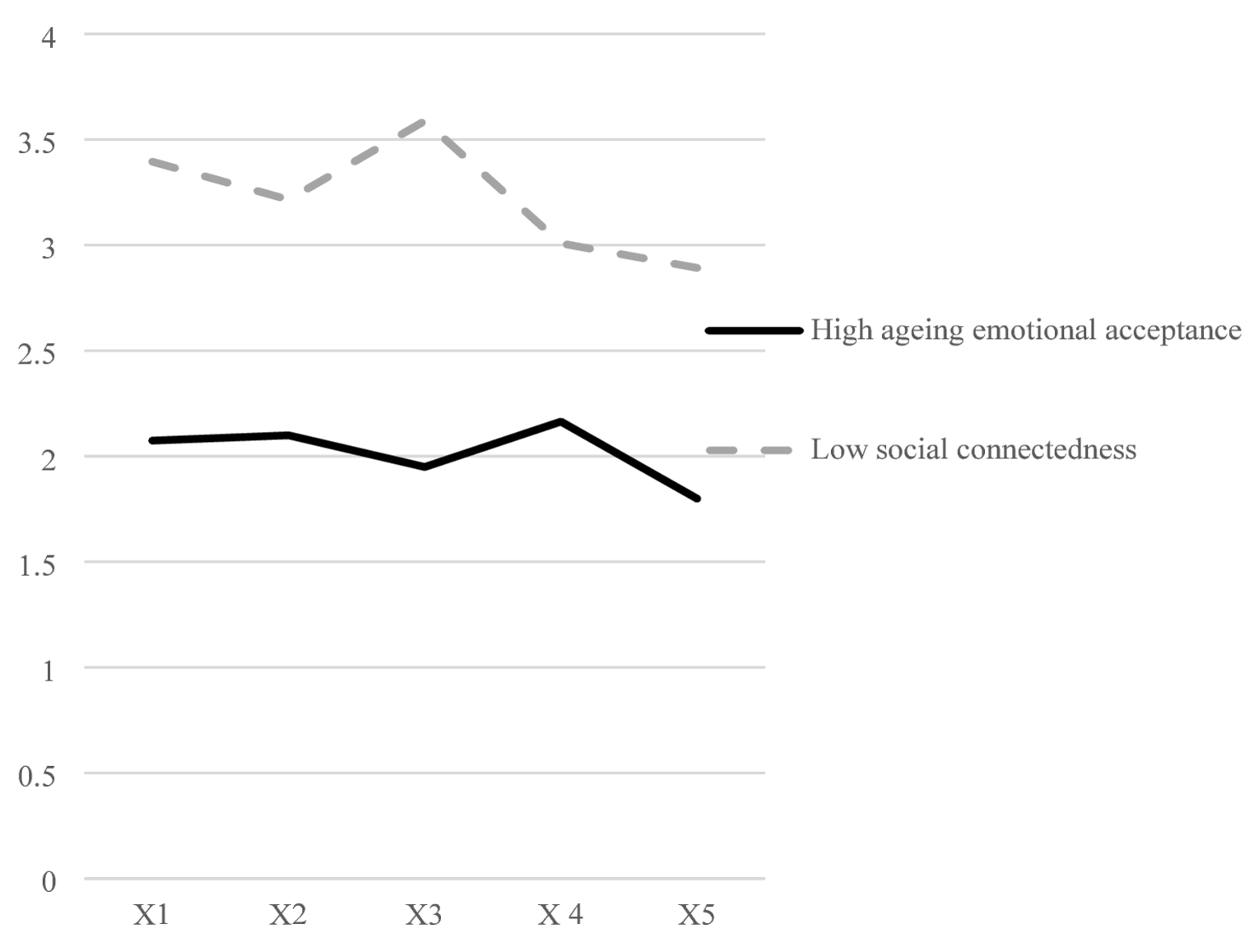
Journal information
- Electronic ISSN
- 2251-6581
- Abstracted and indexed in
-
- Baidu
- CLOCKSS
- CNKI
- CNPIEC
- Chemical Abstracts Service (CAS)
- Dimensions
- EBSCO
- EMBASE
- Emerging Sources Citation Index
- Gale
- Google Scholar
- IFIS Publishing
- Naver
- OCLC WorldCat Discovery Service
- Portico
- ProQuest
- PubMedCentral
- Reaxys
- SCImago
- SCOPUS
- Semantic Scholar
- TD Net Discovery Service
- Wanfang
© Tehran University of Medical Sciences